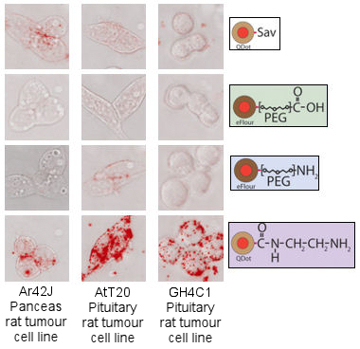
As discussed in the
introduction to cell biology section, cells are very complex micron sized living entities filled with many complex systems to keep them alive and working. In a cell protein synthesis, folding and interactions use protein nanomachines that are almost infinitely more complex than anything that can be made synthetically, and all done at length scales smaller than can be produced by almost any nanotechnology protocols to date.
Hence, nanotechnology in biology is mostly quite simple at the current time as the field is relatively young. The project described here, focused on studying the non-specific internalisation of quantum dots into living cells, represents just such a simple set of experiments that lead to complex results requiring some thought to understand. However, without this basic knowledge it is difficult to extend to more complex ideas. Quantum dots are simple synthetic nanostructures made up of a small crystal of semiconducting material. At the nanometre scale these dots confine electrons as if they were artificial atoms through the process of quantum confinement - all matter can be viewed as waves at the small scale and the electrons in the dot are forced to oscillate at a wavelength given by the dots size in much the same way as a bell will oscillate at one frequency, given by its size. If the electrons in the dot are excited by blue light, they will subsequently decay and give off light of a certain frequency, given by the dots size. In the same way that different sized bells give different sounds, dots of different sizes will emit light of different colours, and it is this property that makes quantum dots very appealing for biology - a large number of different sized dots can be make, all of different colours, and each can be tagged to a different part of a cell so lots of processes can be viewed at once. This was the reason that for our experiments we used quantum dots to look into certain cellular properties. However, being new to biology, we found a number of problems. The first figure shows different quantum dots (coloured red), all bought from major life science companies, with different chemicals on their outer surfaces, as represented on the right side of the figure. These where simply incubated with three different types of cells, indicated on the bottom of the figure, to see what would happen before we started full experimental procedures. As can be seen there is a great deal of variety between the different surface types, with quantum dots sometimes entering cells in vast numbers and sometimes not entering at all.
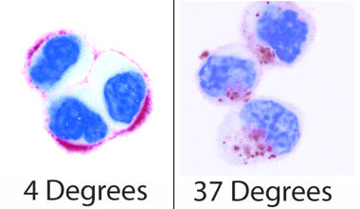
Clearly before even the simplest of experiments using nanoparticles and cells can be done there are a number of questions. Fortunately, the answers to many of these questions have been published over the years, however, the answers are scattered throughout the fields of cell biology, gene transfection, drug deliver and nanoparticle research.
Going back to the very beginning there are some simple experiments to start to understand better the processes by which material enters cells. The first of which is shown in the next figure. This again shows quantum dots (red) incubated with cells (here the nucleus of the cell has been stained blue for ease of viewing), but at two different temperatures. What is clear is that at 4 degrees the quantum dots sit around the edges of the cells while at 37 degrees they are situated inside the cells. At 4 degrees the cell can no longer perform any processes, and so is effectively asleep, while at 37 degrees it is at its most active. Hence these pictures show that the quantum dots enter the cells through processes the cells control. A further test is to feed the cells sucrose before the experiments, and again it is found this stops the quantum dots entering the cells. This tests the method by which the cells internalise the quantum dots, and shows that they enter via entities known as clathrin vesicles. These are the most common type of vesicles the cell uses to move material through the cell membrane, and have been extensively studied. And so, while we say that quantum dots are non-specifically entering the cells (the cell has no use for them, and does not actively try to collect them), they are still brought in through a specific and well controlled pathway.
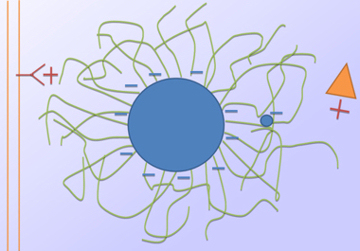
With this understood, various properties of the quantum dots, and in fact any nanoparticles, can be considered to understand the various amounts of internalisation seen in the first figure. It is found that charge is the most important factor. To understand why again the process by which things normal enter cells must be considered. This is done via a process known as receptor mediated endocytosis. All things the cells want are made of proteins, which are made from sequences of amino acids. Each protein has a unique sequence of these amino acids, and special receptor molecules on the cell's membrane are designed to recognise these different sequences. When the cell needs a specific protein, it sends these receptors to the membrane, and when the receptor recognises the protein it is designed to find, it binds to it, and then sends a signal to the cell. The cell then sucks the receptor and the protein into the cell by making a vesicle, which is a small bubble that is pinched off from the membrane and moves into the cell where it is dismantled and the required protein is extracted. As with everything in cell biology this is a very complex process, however we can simply understand that anything that can trigger a receptor on the cells surface will be sucked into the cell via a vesicle. Amino acids are electrically charged, and the binding is done via electrostatic interactions, so there are two possible means by which charged nanoparticles can enter the cell. The first is a direct method where the charged particle can trigger the receptor. The second is less direct and requires the charged particle to attract proteins sitting in the medium surrounding the cells, these proteins then interact with the receptors, and since the nanoparticles are stuck to the proteins they are internalised at the same time. Of the two possible methods both probably occur, but the second is of greatest importance. So, it is understood that the more charged a particle the more easily it will be internalised into a cell, which is consistent with our results. The next observation is that when a certain molecule called PEG is used to coat the quantum dots the amount of cellular internalisation is reduced to very low levels. Again, here charge is all important. As shown in the sketch PEG is a long chain molecule with no charge (green). When lots of it is present on the surface of the quantum dot it physically separates the charged surface of the dot from the outside world. This all but stops both receptors and proteins from being attracted to the charged surface, and so the interactions are reduced and the quantum dot is no longer internalised. It can also be noted that charges on the end of the PEG molecule can become lost in the tangle of molecules, again reducing any interactions.
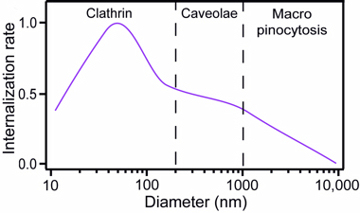
Finally, it is interesting to consider the influence particle size has on the rate of internalisation. While the quantum dots used in our study are all about 20 nm in size, when they are added to the cell culture medium there tends to be some particle aggregation, so the cells see particles of a larger size. While it is not possible for us to directly study the influence of size, it is possible to compile multiple literature sources on the influence of size over internalisation, and look for trends over a full range of sizes. This gives rise to the final graph in this section and shows three approximate regimes. It should be noted that this is very much an approximation, and depends on the type of cells used as well as other factors such as the environmental conditions; however, the general trend is true for most usual situations. In the small-scale regime clathrin vesicle internalisation is the dominant mechanism, such as would also found for the 20 nm quantum dots. Here there is a peak in the internalisation rate for particles of approximately 50 nm in size. The reason for this is that for a vesicle to form, in reality multiple receptors must be triggered. For particles of 50 nm size a single particle can trigger enough receptors to initiate internalisation; however, when the particles are smaller than this, multiple particles are needed in close proximity so the rate drops. When particles are larger than 50 nm, they use up more receptors than is necessary, and since there are only a certain number of receptors available, this also has the effect of reducing the internalisation rate. Clathrin vesicles can only form up to about 200 nm in size, and so for particles larger than this the cell finds it more difficult to internalise them, leading to a drop in the internalisation rate. Up to about one micron in size caveolae vesicles can internalise particles, these are much the same as clathrin vesicles but can grow to a larger size and are formed less often, causing a reduced rate. Above one micron in size cells find it almost impossible to internalise particles, however under certain conditions the effect of macro-pinocytosis can occur where the cell membrane ruffles and larger amounts of external material enter the cell via a very large vesicle.
These observations bring a reasonable level of understanding to the observed interactions between quantum dots and cells, and while are not new, can be of use to other physicists wishing to have a starting point to move into cell biology, and to aid in the development of nanoparticles for the study of
receptor mediated endocytosis .
Papers relating to this work:
Non-specific cellular uptake of surface-functionalized quantum dots
T.A. Kelf, V.K.A. Sreenivasan, J. Sun, E.J. Kim, E.M. Goldys and A.V. Zvyagin
Nanotechnology.
21, 285105 (2010)
Pharmacological Characterization of a Recombinant, Fluorescent Somatostatin Receptor Agonist
V.K.A. Sreenivasan, O.A. Stremovskiy, T.A. Kelf, M. Heblinski, A.K. Goodchild, M. Connor, S.M. Deyev, and A.V. Zvyagin
Bioconjugate. Chem.
22, 1768-1775 (2011)
Barstar:barnase - a versatile platform for colloidal diamond bioconjugation
V.K. . Sreenivasan, E.A. Ivukina, W. Deng, T.A. Kelf, T.A. Zdobnova, S.V. Lukash, B.V. Veryugin, O.A. Stremovskiy, A.V. Zvyagin, and S.M. Deyev
J. Mat. Chem.
21, 65 (2011)
A modular design of low-background bioassays based on a high-affinity molecular pair barstar:barnase
V.K.A. Sreenivasan, T.A. Kelf, E.A. Grebenik, O.A. Stremovskiy, J.M. Say, J.R. Rabeau, A.V. Zvyagin, S.M. Deyev.
Proteomics.
13, 1737-1443 (2013)